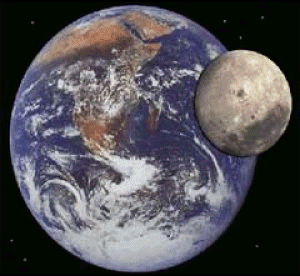
Newton proposed that every object with mass exerts a gravitational pull or force on every other object with mass in the universe. Well, the earth is much more (80more) massive than the moon, which is why the moon orbits us, and not we it. (If you want to get technical, we both actually orbit an imaginary point called the center of mass.) However, the moon is sufficiently massive to make the effects of its gravitational field felt on the earth.
Anyone who lives near the ocean is familiar with tides. Coastal areas experience 2 high and 2 low tides within any 24-hour period. The difference between high and low tides is variable, but, out in the open ocean, the difference is somewhat more than 3 feet. If you’ve ever lifted a large bucket of water, you know how heavy water is. Imagine the forces required to raise the level of an entire ocean 3 or more feet! What force can accomplish this?
The tidal force of gravity exerted by the moon on the earth and its oceans. The moon and the earth mutually pull on each other; the earth’s gravity keeping the moon in its orbit, the moon’s gravity causing a small deformity in the earth’s shape.
This deformity results because the moon does not pull equally on all parts of the earth. It exerts more force on parts of the earth that are closer, and less force on parts of the earth that are farther away. Just as Newton told us: Gravitational forces depend on distance. These differential or tidal forces are the cause of the earth’s slightly distorted shape—it’s ovoid rather than a perfect sphere— and they also make the oceans flow to two locations on the earth: directly below the moon, and on the opposite side. This flow causes the oceans to be deeper at these two locations, which are known as the tidal bulges. The entire Earth is pulled by the moon into a somewhat elongated—football—shape, but the oceans, being less rigid than the earth, undergo the greatest degree of deformity.
Interestingly, the side of the earth farthest from the moon at any given time also exhibits a tidal bulge. This is because the Earth experiences a stronger gravitaional pull than the ocean on top of it, and the Earth is “pulled away” from the ocean on that side. As the Earth rotates beneath the slower-moving moon, the forces exerted on the water cause high and low tides to move across the face of the earth.
The tides of largest range are the spring tides, which occur at new moon, when the moon and the sun are in the same direction, and at full moon, when they are in opposite directions. The tides of smallest range are the neap tides, which occur when the sun and the moon are at 90 degrees to one another in the sky. Tides affect us every day, of course, especially if you happen to be a sailor or a fisherman. But even if you live high and dry in Kansas or Nebraska, say, tides (and the moon) still affect you. Every day, the earth is spinning a little slower on its axis because of the moon. The earth’s rotation is slowing down at a rate that increases the length of a day by approximately 2 milliseconds (2/1,000 of a second) every century. Over millions of years, though, this slowing effect adds up. Five hundred million years ago, a day was a little over 21 hours long, and a year (1 orbit of the sun) was packed with 410 days. When a planetesimal plowed into the earth early in the history of the solar system, it was rotating once every 6 hours. (And you think there aren’t enough hours in the day now!)
How does this happen? Well, let’s think again of why tides occur. The moon’s gravity causes two bulges to form in the earth’s oceans, and the earth rotates (once every 24 hours) beneath that bulge. As the earth spins, friction between it and the oceans tends to pull the high tide ahead, so that the “bulge” actually leads the position of the moon overhead.
With the ocean’s bulge thus slightly ahead of the moon’s position, the moon’s gravity exerts a force that tends to slow rotation. Eventually, the earth’s rotation will slow sufficiently to become synchronized with the orbit of the moon around the earth. When that happens, the moon will always be above the same point on Earth, and the earth’s rotation period will have slowed (billions of years from now) from its present 24 hours to 47 days.
But that is only half of the picture. The earth can’t be slowing down without something else speeding up (as a result of one of the fundamental conservation laws of physics). What’s speeding up? The moon. And what does that mean? That it’s spiraling away slowly, and getting smaller and smaller in the sky.